The challenge to observe and analyze living cells
The acquisition of information about the “molecular state” of a sample already is a hard task in fixed cells or tissue. This becomes even harder, if the information has to be acquired in real-time, as the cells during an experiment have to be functioning as naturally as possible. Additionally, a high amount of information has to be sampled in a relatively short time, as many events only last seconds or even milliseconds (e.g. changes in cellular ion levels).
One approach to address these challenging demands are certain optical methods that are collectively called live-cell imaging. Live-cell imaging allows for investigation of dynamical physiological processes in living cells instead of giving a “snapshot” of a cell’s current state. It turns Snapshots to movies. Live-cell imaging provides spatial and temporal information of dynamic molecular events in single cells, cellular networks (in situ) or even whole organisms (in vivo). These features make live-cell imaging a requisite technique for addressing physiological questions in cell biology, cancer research, developmental biology and neuroscience.
In recent years substantial advances in electronics, optics and biochemistry made live-cell imaging easily accessible for scientists. Using optimized microscopes, specialized light sources, high speed cameras, highly sensitive detectors and exclusively developed fluorescent markers, live-cell imaging methods nowadays provide both - a technically mature and still innovative toolsets to address the challenging demands of investigating single cells or whole cellular networks on a molecular level in real-time.
Live-cell imaging of cells with a mitochondrial marker (MitoRed®) and a fluorescent calcium dye (Fluo-4)
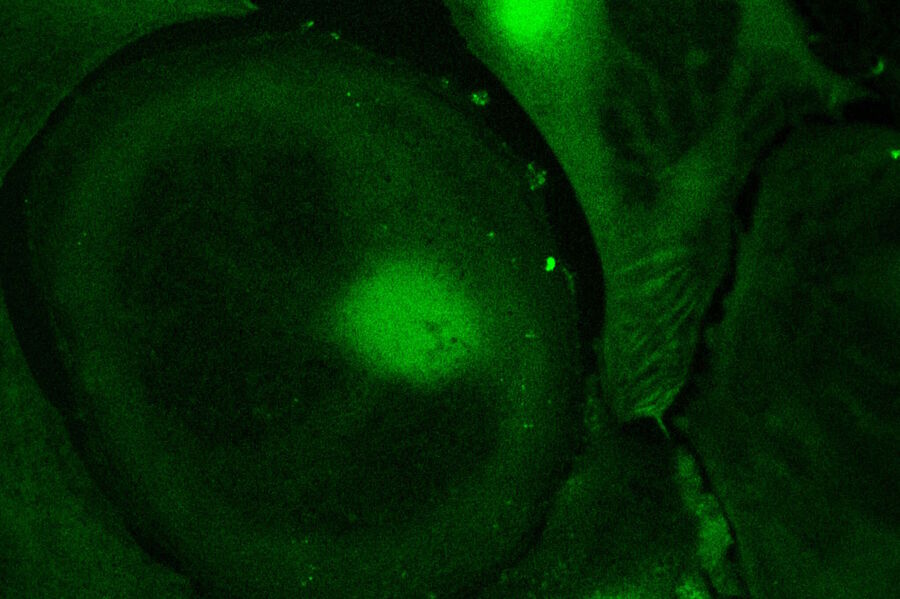
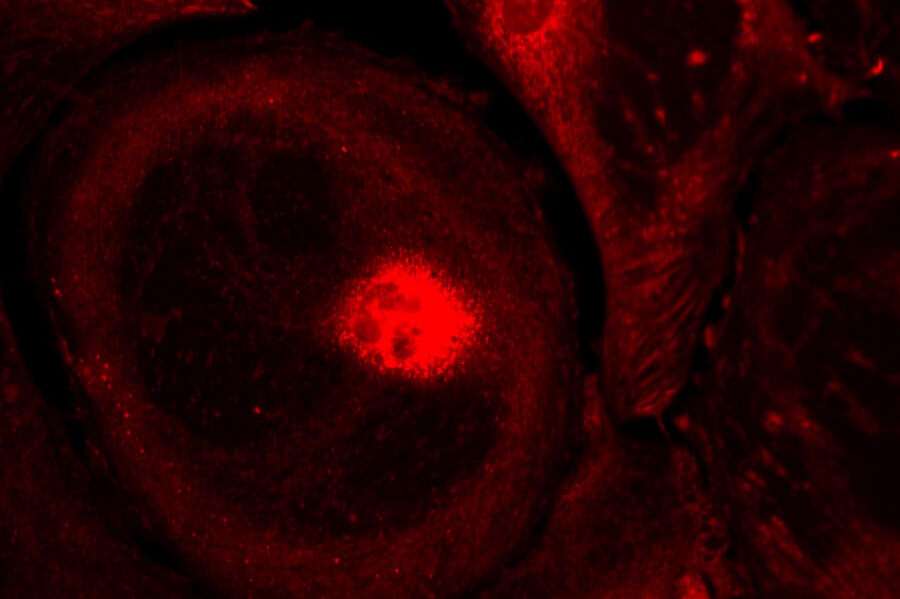
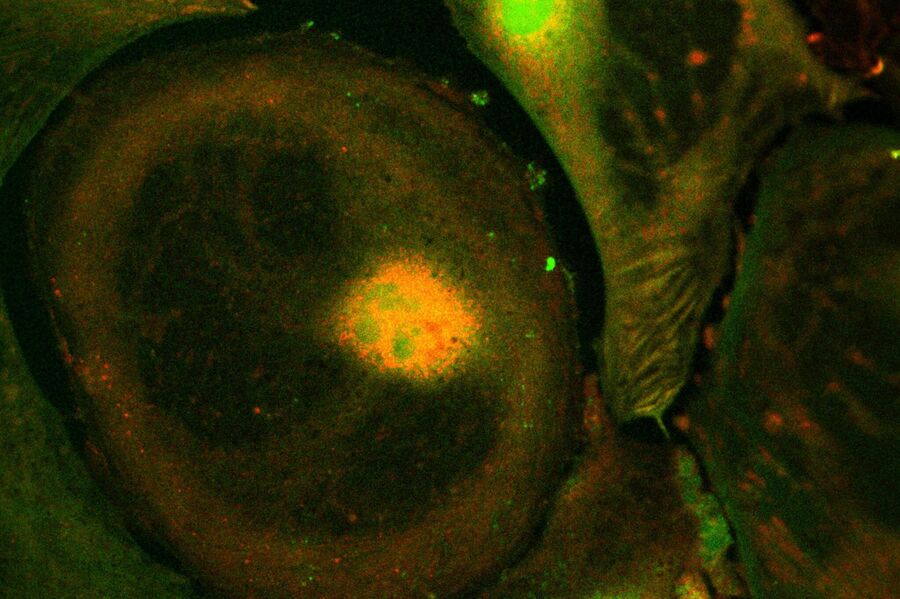
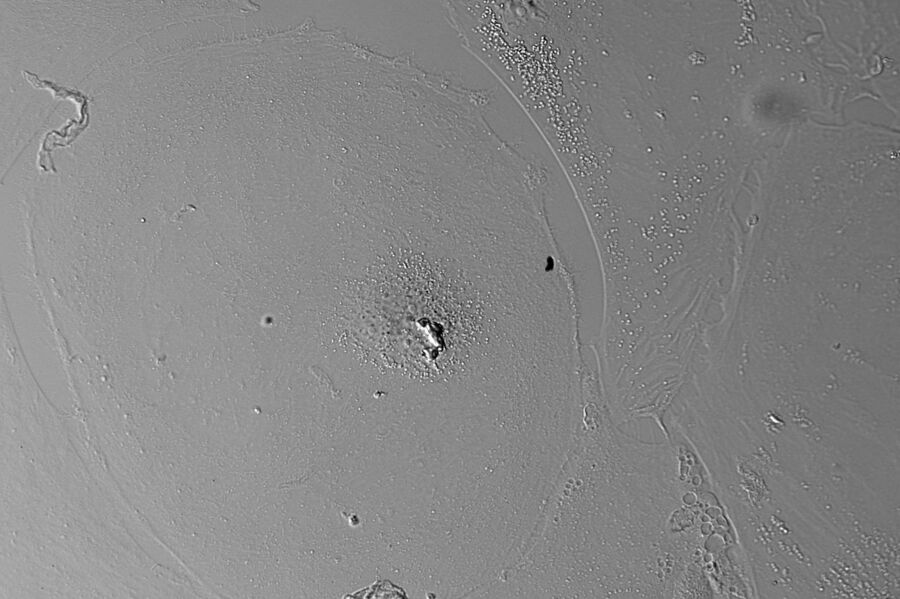
Images acquired with a confocal microscope Leica TCS SP5 (DMI6000 CFS) and Leica